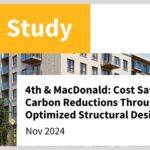
4th & MacDonald: Cost Savings and Carbon Reductions Through Optimized Structural Design
November 25, 2024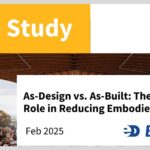
As-Design vs. As-Built: The Builder’s Role in Reducing Embodied Carbon
February 24, 2025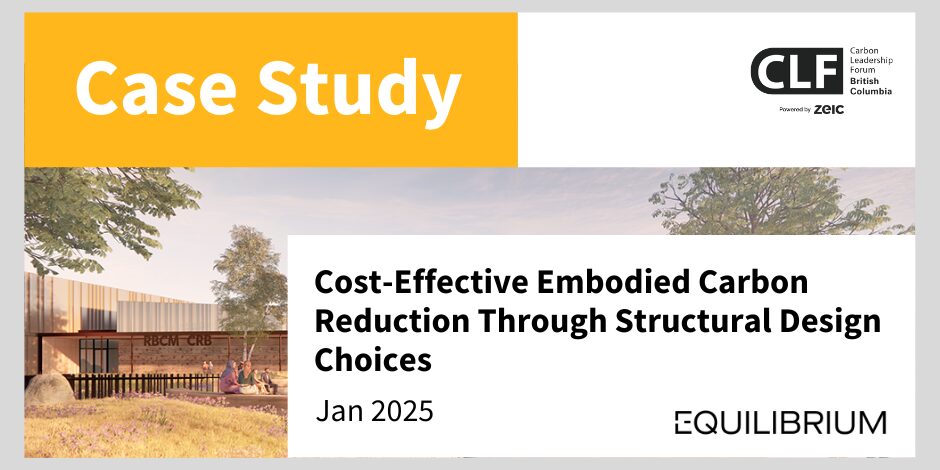
Introduction: Embodied Carbon in Context
In order to avoid the worst effects of climate change, countries and industries alike have pledged to significantly reduce carbon emissions and achieve the science-based targets set by the United Nations Intergovernmental Panel on Climate Change. For the built environment, this proposes construction of new buildings and infrastructure achieving net zero carbon emissions by 2050 whilst hitting intermediate milestones including a 40% reduction in emissions by 2030[1].
The term ‘embodied carbon’ refers to the greenhouse gas emissions associated with the production, use and disposal of materials used in construction. As renewable energy adoption becomes an increasingly common strategy to reduce operational carbon, the embodied carbon in construction materials has begun to dominate the overall carbon emissions of the built environment.
The embodied carbon of construction materials comes mostly from emissions at the production stage. Reducing embodied carbon can therefore be achieved in two ways: reducing the volume of material used in construction, and using materials with lower embodied carbon emissions associated with their supply.
Sources of embodied carbon vary by project. Typically, over 50% of the upfront embodied carbon is associated with the construction of the foundations and primary structure, with the façade, MEP system, interior fit-out and finishes accounting for the remainder.[2] New buildings typically fall within a range of 100-500 kgCO2e/m2 (for Part 3, including foundations, primary structure and façade). In a recent study of more than 50 projects by the City of Vancouver, 75% of new buildings were found to be below 360kgCO2e/m2, with one-third of all concrete buildings below 300kgCO2e/m2.[3][4]
Embodied carbon limits are being introduced into legislation in North America. Upcoming code changes in Vancouver will encourage efficient structural design aimed at remaining below a threshold of 360kgCO2e/m2 or demonstrating, through active design decisions, a reduction of 10% compared to a baseline building[5]. In the US, California recently introduced code clauses that also require building designers to use low-carbon materials and remain within similar reductions based on a building’s useable floor area.[6]
Demonstrating that a building achieves such limits should be seen as simple. The intent of such policies is to act as a stepping stone, mobilizing an industry and shifting the culture so that more stringent reductions necessary by 2030 and 2050 are achievable.
The Evolving Role of the Structural Engineer
Understanding embodied carbon and assisting Life Cycle Analysis (LCA) is now part of a structural engineer’s role. Many structural engineering firms have made public commitments to reduce carbon emissions on their projects; in BC, a total of 12 structural consultancies have each signed up to industry-wide carbon reduction initiatives, SE2050 and Canadian Engineers Declare.[7][8]
The basic effort required by a structural engineer to provide member sizing and material specifications to the LCA assessor is similar to that typically seen for interfacing with a QS on all but the most complex Part 3 buildings. However, many consultants choose to exceed this, recognizing their value and the opportunity for structural engineers to reduce global emissions through design decisions.
The following report includes a non-exhaustive list of structural design strategies to help limit embodied carbon on projects in British Columbia. For greater reductions, additional measures that consider building form, mechanical systems and interior finishes may be considered as part of a holistic LCA assessment.
Structural Design Strategies – Scheme Design
Figure 1 shows the design hierarchy recommended by bodies such as EGBC, SE2050 and the IStructE to ensure efficient structural design and maximize potential reductions in embodied carbon. Design teams should understand the motives at the inception stage, to establish the suitability of a project and client appetite for deep carbon reductions.
Where building nothing or building less through re-use of existing building stock or the reduction in new building area is not feasible, choosing to build smart provides numerous options; reducing both cost and carbon is possible before specifying sustainable or innovative materials.
Structural engineers should be encouraged to challenge the project brief and work in collaboration with the architect. Adjusting the building geometry can realize the biggest savings in structural carbon.

Examples of design strategies include:
- Reducing or removing basement volumes and challenging required parking areas.
- Aligning vertical structure to avoid large transfer beams and slabs.
- Shortening column grids and floor spans to reduce slab depths and save material.
- Reducing specified floor loading, where feasible, to save on floor, column wall and foundation structure.
- Changing floor or beam framing geometry to maximize efficient use of materials.
Figure 2 shows potential for embodied carbon reductions from the strategies above for a typical concrete building. Similar tactics also exist for buildings constructed in steel and mass timber.
By asking the right questions early in the design process, structural engineers can save significant amounts of carbon, improve design efficiency and save cost, helping streamline the process for an optimized solution. A well-managed design team with a culture of open collaboration between the Client, Design Consultants and Contractor is also key to aligning cost, carbon and program savings in a project.
The strategies above apply universally regardless of location and choice of material. With respect to the Vancouver Building Bylaw, such strategies may achieve absolute limits on carbon intensity or act as a framework to inform design decisions.
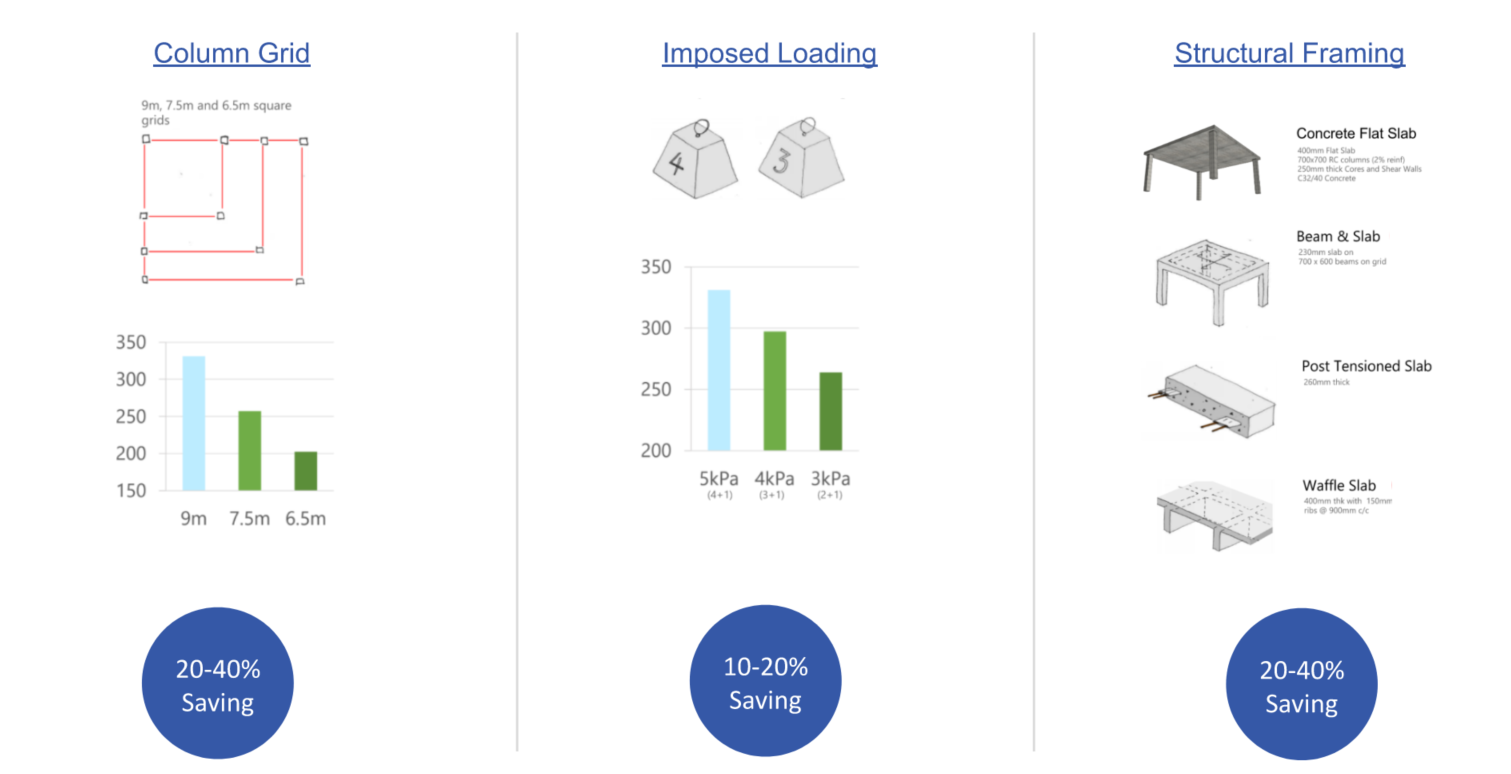
Structural Design Strategies – Specification
Specifying low embodied carbon materials should always come after opportunities to reduce material volumes and new building volumes have first been explored.
Low-carbon materials are a vital resource for deeper reductions in embodied carbon. Alternative materials may come with cost premiums and varying levels of carbon impact; however, numerous effective choices are feasible in British Columbia and the Pacific Northwest (PNW) at a low- to zero-cost differential. Choices should be made by a project team and client in collaboration with the structural and sustainability engineer as early as possible in the design process. This helps ensure they are feasible, available and practical.
Examples of low-to-no cost specification options in BC include:
- Specifying GUL concrete (including limestone), resulting in a 5-15% CO2e/m3 saving versus traditional GU concretes, with no cost or curing impact. GUL is now widely supplied as standard throughout BC.[9]
- Setting an overall concrete carbon budget or an average GWP across all concrete mixes. Allowing the Contractor to decide where higher SCM mixes are used is proven to give better outcomes. Slower curing mix options can then be used on non-critical path elements to avoid impact on project schedules.
- Specifying 56-day concrete strengths for foundation and walls elements to reduce cement content and carbon if early age strength gain is not required as based on lower temporary loads.
- Avoiding air-entrained concrete where not required (insulated footings and internal areas) to save approximately 5% CO2e/m3.
- Utilizing reinforcement manufactured in the PNW, and manufactured via the Electric Arc Furnace method, to benefit from a low-carbon electricity grid and short transport distances.[10]
- Utilizing locally sourced mass timber or, where sourced from out of province, sizing timber elements to enable transportation via rail and ship. Projects are advised to limit the impact of transport emissions (stage A4) from trucking, which has five times the impact of rail and ship per kilometer.[11]
In the case of emerging and innovative materials, specification and adoption should be carried out in collaboration with a sustainability consultant to ensure the benefits expected are justified from a detailed LCA. The impact of long-distance transportation should be included in the decision-making process as well as end-of-life impacts for complex or composite materials.
Example: Royal BC Museum Collections & Research Building
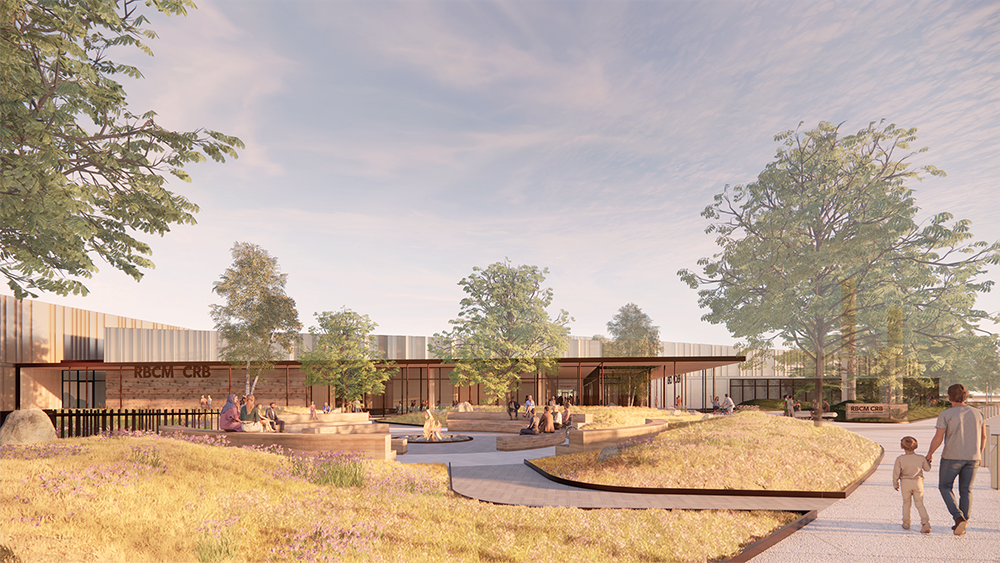
For the new 15,200m2 Royal BC Museum (RBCM) Collections and Research building in Colwood BC, EQUILIBRIUM collaborated closely with the concrete supplier and contractor. Maple Reinders was then able to maximize the use of SCMs to reduce carbon in the concrete mixes. The supplier was able to provide a 30MPa concrete mix including SCMs, with a 20% reduction in carbon (230kgCO2e/m3) at no cost or program impact, and a 40% GWP reduction mix (170kgCO2e/m3) at 5% cost premium utilizing increased levels of slag SCM.
The Long-Term View
The strategies listed above are appropriate and actionable on projects today, where limiting embodied carbon is a requirement or goal.
For many leading authorities in North America, incoming regulations such as the City of Vancouver’s VBBL 2025 & California’s CALGreen, have initially adopted relaxed targets that are simple to achieve, to act as guidance for industry.
To achieve the deeper UN decarbonization goals agreed to by governments around the world including Canada and the United States, subsequent revisions to these codes will require increasingly stringent carbon limits for 2030 and beyond.
Aligning with the proposed trajectory requires innovation from the construction industry, both in terms of culture and technology. Areas most likely to see this include:
- Building retrofit and strengthening. By extending the service life of existing buildings, owners can avoid higher embodied carbon emissions associated with new construction. For projects in British Columbia, updated code provisions that aim to facilitate re-use and seismic retrofitting would make this strategy more achievable and economical in practice.
- Structural material re-use. Direct re-use of materials including steel sections, concrete aggregate and aluminum façade panels has the potential to save significant carbon compared to manufacturing these as new. Wider adoption will require a culture shift from demolition to deconstruction, with clarity on available materials through an accessible inventory.
- Material innovation. Increased supply, recovery and use of SCMs for concrete, with the adoption of Carbon Capture and Storage (CCS) technologies in concrete and steel production both reduce embodied carbon. Coupled with the use of renewable energy sources in material production and innovation in shipping and transportation, this will enable efficient movement of new materials across North America with low carbon impacts.
- Building massing changes. A shift in design culture away from the use of high embodied carbon elements, such as podium transfer slabs and large basement structures, will help reduce embodied carbon.

As shown, in order to achieve fully net zero embodied carbon buildings by 2050, the industry will need to adopt a combination of current and future technologies. Both strategies are needed in parallel with new design approaches and evolving legislation. The role of structural engineers in ensuring that the long-term carbon reduction targets proposed can be achieved in practice cannot be understated.
References
References
[1]: https://www.ipcc.ch/2022/04/04/ipcc-ar6-wgiii-pressrelease/
[2]: Embodied Carbon Primer, LETI, 2020
[3]: Vancouver Building By-law, 2019
[4]: City of Vancouver - Rezoning Data, 2017-2023
[5]: Embodied Carbon in Vancouver Building Bylaw 2025 (Presentation), Z Teshnizi, 2024-05-06
[6]: California Green Building Standards Code Supplement, 2024-07-01
[7]: https://se2050.org/signatory-firms/
[8]: https://ca.engineersdeclare.com/
[9]: Concrete BC, Industry-wide Ready-Mixed Concrete EPD, 2022
[10]: Steel Reinforcing Bar and Merchant Bar Products EPD, Nucor, 2022
[11]: https://www.epa.gov/climateleadership/ghg-emission-factors-hub